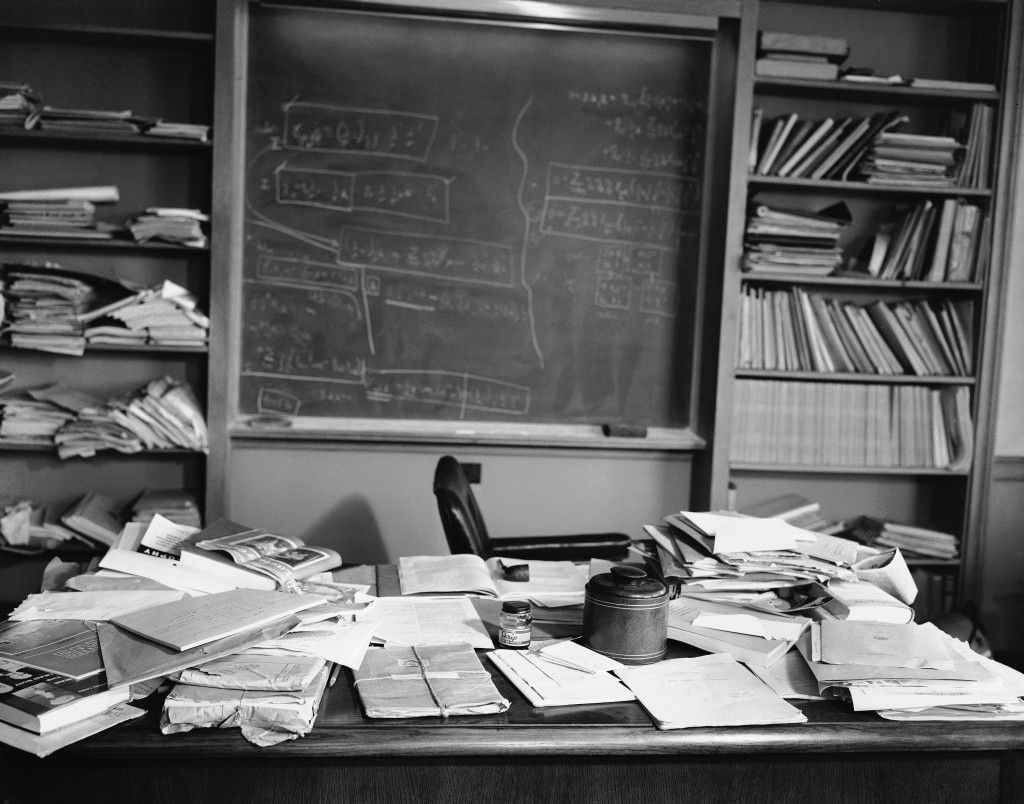
Research
Biological cells are more than genes and networks of interacting proteins; they are also physical objects, which, in addition to sensing chemicals, can also sense physical and mechanical stimuli. With a distinction between living and non-living matter rather blurry, my vision for biological physics is the extension of the historic successes of physics to living, biological systems. The goal is to identify overarching principles to simplify and understand biological signaling, complexity, optimality, and information processing. Such understanding is expected to also have drastic impact on biomedical applications.
Nonequilibrium processes underpinning life
Cells are ‘open systems’, characterised by fluxes of energy and matter across their membranes. This conversion of free energy into heat and waste products allows them to maintain, grow, and reproduce. While modern cell biology can describe the underlying molecular processes extremely well, little is known about how such ordered systems could have emerged in the first place. Consider a traditional ‘closed system’. The second law of thermodynamics says that after waiting sufficiently long the resulting state is one of maximal disorder or entropy. However, how is this maximum entropy state achieved? Does the system follow the quickest path, which produces as much entropy as possible, or does it equilibrate as slowly as possible, following a minimum entropy production principle? My group addresses such thermodynamic selection principles using methods from stochastic thermodynamics.iological cells are more than genes and networks of interacting proteins; they are also physical objects, which, in addition to sensing chemicals, can also sense physical and mechanical stimuli. With a distinction between living and non-living matter rather blurry, my vision for biological physics is the extension of the historic successes of physics to living, biological systems. The goal is to identify overarching principles to simplify and understand biological signaling, complexity, optimality, and information processing. Such understanding is expected to also have drastic impact on biomedical applications.

Physical limits of sensing
In 1977, physicists Howard Berg and Edward Purcell published their results on a fundamental biological problem of sensing. The question they addressed was how accurately a biological cell, viewed as a tiny measurement device, can sense its chemical environment in a given amount of time using cell-surface receptors. In essence, the message of the paper was simple: sensing in the microscopic world boils down to counting molecules, which arrive at the cell surface by diffusion. Humans encounter a similar limit when we try to see in the near dark as our photoreceptors count single photons. However, Berg and Purcell assumed that the best a cell can do is to time average the noisy signal. Work in my group addresses the roles of receptor clustering, energy consumption, and memory in increasing the accuracy of sensing beyond the Berg and Purcell limit.

Information processing in bacteria
Sensory systems have evolved to respond to input stimuli of certain statistical properties, and to reliably transmit this information through biochemical pathways. Hence, for an experimentally well-characterised sensory system, one ought to be able to extract valuable information about the statistics of the stimuli. Previously, we predicted the chemical gradients chemotactic Escherichia coli cells typically encounter in their natural environment, such as the human intestines. Initially, we used phenomenological laws known from neuroscience such as Weber's law, Weber-Fechner law, and fold-change detection. More recently we began to apply information theory and simulations of swimming bacteria, considering limitations of information transmission from both cell-external and internal noise. Future work will consider time-dependent problems based on trajectories of swimming bacteria, linking information transmission with optimal behaviour and energy consumption.
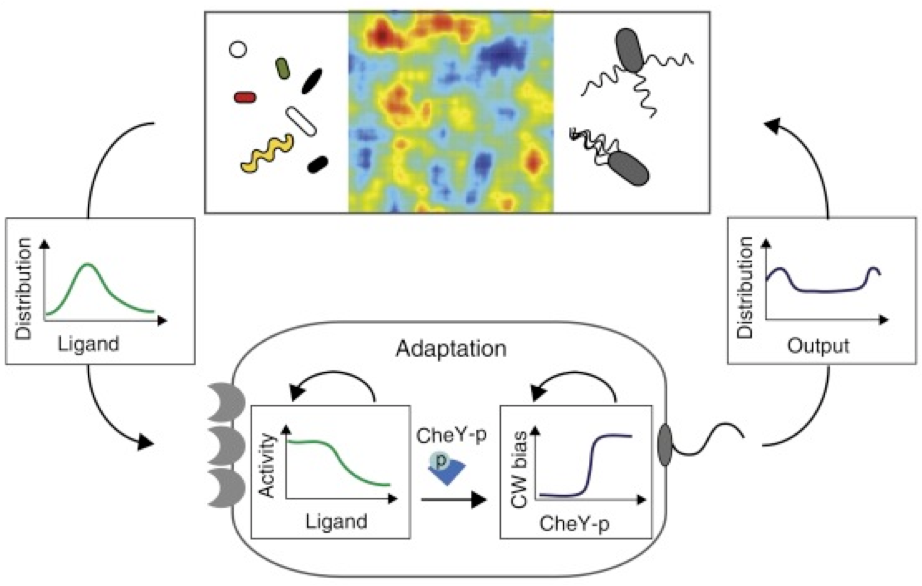
Cell shape and behaviour of eukaryotic cells
The behaviour of an organism often reflects a strategy for coping with its environment. Such behaviour in higher organisms can often be reduced to a few stereotyped modes of movement due to physiological limitations, but finding such modes in amoeboid cells is more difficult as they lack these constraints. In previous work, we examined cell shape and movement in starved amoebae during migration toward a chemoattractant in a microfluidic chamber. We showed that the incredible variety in cell shape across a population can be reduced to a few modes of variation. Interestingly, cells use distinct modes depending on the applied chemical gradient, with specific cell shapes associated with shallow, difficult-to-sense gradients. Modelling and drug treatment reveals that these behaviours are intrinsically linked with accurate sensing at the physical limit. Since similar behaviours are observed in a diverse range of cell types, we propose that cell shape and behaviour are conserved traits. Ongoing work aims to quantify trajectories of cell shape and to link them to strategic behaviour, e.g. using techniques from statistical physics and statistical inference.
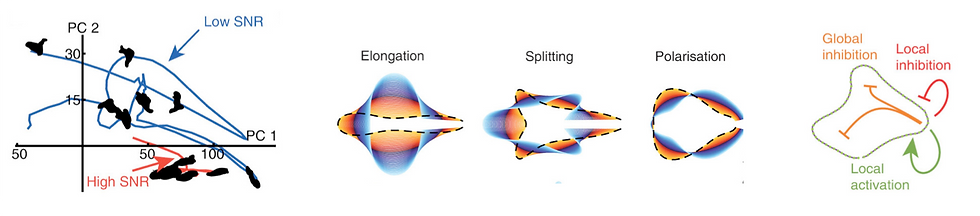
Engulfment across the kingdoms of life
Phagocytosis is the fundamental cellular process by which eukaryotic cells bind and engulf particles by deforming their plasma membrane. Particle engulfment involves particle recognition by cell-surface receptors, signalling and remodelling of the actin cytoskeleton to guide the membrane around the particle in a zipper-like fashion. The signalling complexity is daunting, involving hundreds of different molecular species during the initial stages of engulfment. Despite the signalling complexity, phagocytosis also depends strongly on simple biophysical parameters, such as particle shape, orientation and stiffness, largely independent of type of cell or particle. We argue that these emergent, universal features are particularly important to address in order to explain this evolutionary well-conserved and robust mechanism. In addition we also study engulfment in sporulating bacteria. Here, the cell performs asymmetric cell division. Subsequently, the dividing septum is thinned and remodelled, resulting in the larger mother cell engulfing the smaller forespore for spore maturation and ultimately spore release. The physical mechanisms are very little understood, and studying bacteria and eukaryotes may provide general principles of engulfment.

Collective behaviour at multiple scales
Biological systems are often conceptualised as networks of interacting genes and proteins. Nevertheless, a simple analysis of the fundamental genetic programs is often not sufficient to explain higher-level functions such as multi-cellular aggregation, tissue organisation, and embryonic development. Furthermore, various aspects of these processes are often emergent, irrespective of their underlying microscopic details. As such, related issues arise in groups of individuals, e.g. in animals and humans. Thus tackling these problems requires a holistic approach, aimed towards discovering general overarching principles in biological systems. Indeed, in the past few years, larger scale experiments allowed the construction of dynamic and statistical-mechanics models of biological systems directly from real data, producing immense progress in our understanding of emergent collective behaviour in biology. In my group, we investigate collective behaviour twofold: First, we study starved amoebae, which aggregate artfully in form of patterns for fruiting body and spore formation. Second, we compare social and asocial nematode worms and study how collective behaviour influences feeding and ultimately fitness.
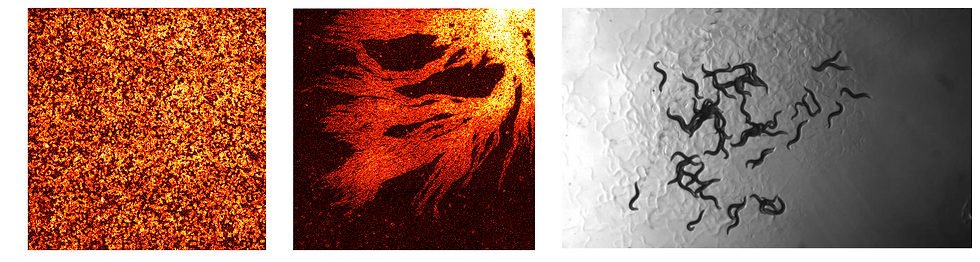